Authors: Magdalena Pappenreiter, Hubert Schwarz, Bernhard Sissolak, Alois Jungbauer, Véronique Chotteau.
First published: 26 March 2023 – https://doi.org/10.1002/jctb.7386
Abstract
BACKGROUND
Tangential flow filtration (TFF) systems are widely used cell retention devices in perfusion cultures, but significant challenges occur with their prolonged operation. A well-known and common issue includes membrane fouling, which leads to reduced permeate flow and increased product retention. The fouling behavior in hollow fibers have been studied widely and the application of alternating flow profiles, cell lysis, shear stress, and membrane pore size have been found to be major contributors to this phenomenon.
RESULTS
In this work, different process set ups and conditions were tested using magnetic levitation pumps for low shear TFF systems in small-scale perfusion bioreactors (200 mL). A novel concept based on the application of reverse flow across the hollow fiber using two magnetically levitating pumps was validated with a Chinese Hamster Ovary cells (CHO) cell line producing a recombinant monoclonal antibody. Product sieving could be improved by 30% when a dynamic recirculation flow was applied. Furthermore, minimal product retention was achieved by reversing the flow of two alternating pumps with short cycle times. Besides this, a correlation was found between the passage of high molecular weight species to the harvest stream of the perfusion process and the flow direction, as well as the degree of product sieving.
CONCLUSION
TFF with a reverse flow is a valuable alternative to an alternating tangential flow (ATF) system for overcoming antibody retention and it can be used at various scales and at a constant bioreactor volume. The comparison of ATF and TFF showed differences in product yield and purity and is, therefore, an important point for process design. © 2023 The Authors. Journal of Chemical Technology and Biotechnology published by John Wiley & Sons Ltd on behalf of Society of Chemical Industry (SCI).
INTRODUCTION
Continuous intensified bioprocesses offer the possibility of long-term operation under steady state conditions with increased operational flexibility, higher productivity, and reduced floorspace requirements compared to legacy fed-batch processes. Direct integration of a high cell density perfusion process with a continuous downstream capture step can save enormous costs. However, a reliable cell retention device is the heart of the perfusion process and must function over a long period of time without tremendous product retention or particle breakthrough in the permeate to allow for continuous harvesting of the target product.
Tangential flow filtration (TFF) technology uses a recirculation device, usually a peristaltic pump, to transport the cell culture parallel to the membrane surface, creating liquid shear to prevent gel layers from forming on the filter surface. However, peristaltic pumps are reported to increase cell lysis due to high shear stress onto the cells. In this aspect, magnetic levitation pumps offer an alternative for low-shear, pulsation free, and continuously controlled flow applications in bioprocessing. The pump impeller is suspended in a sealed housing without contact and is driven by the magnetic field of the motor, resulting in a continuous, uniform flow with extremely low shear to cells. An alternative to unidirectional TFF is the alternating tangential flow (ATF) system, which has gained popularity in recent years and uses a diaphragm pump to move the cell culture back and forth in the hollow fiber. It has been discussed already that this backflushing and regeneration of the hollow fiber results in better product recovery.
Perfusion titers govern the economic and ecological impact of the process. Hollow fiber filtration systems are the technology of choice for cell retention in high density perfusion cell cultures in biomanufacturing. However, they are associated with problems, such as susceptibility to membrane fouling and, consequently, lower product yields in the permeate. Product sieving issues in TFF or ATF directly affect the total product yield. Sometimes, the transmembrane pressure (TMP) in the hollow fiber filter can be affected, resulting in reduced permeate flow and resulting in premature process termination. This is very often the case for cartridges where the hollow fibers are ultrafilters. In addition, variations in the amount of product sieved from the hollow fiber filter can create unstable product concentrations, leading to increased complexity in the integration of the capture step downstream in the perfusion process.
Numerous studies have aimed at determining the underlying causes of membrane fouling to develop principles to overcome product retention. In downstream TFF processes, higher shear rates can advantageously delay membrane fouling; on the other hand, excessive shear stress (by peristaltic pumps) in cell cultures generally causes lower cell viability, which negatively affects product sieving. A few strategies have employed macro-porous filters of pore membranes up to 10 μm (instead of 0.2 to 0.45 μm for micro-filtration) and wide-surface pore microfiltration membranes, making it possible to prevent irreversible fouling due to perfusion of submicron particles through the larger pores. However, downstream of these macro-porous filters, an additional depth filtration step is required to remove small particles from the permeate stream. Other studies have proven that ATF is the method of choice for increasing sieving efficiency in the production of recombinant proteins while at the same time achieving high cell densities. It was also shown that optimized process operations with defined crossflow ranges further reduce product retention and column clogging in ATF-based perfusion cultures. Besides that, studies on mechanistic models for membrane fouling in ATF and TFF perfusion cultures reveal the theory of cake formation causing the product retention problem, along with other factors, such as filter material, pore sizes, antifoam, shear stress, and aggregation propensity of the product. The effects of filter materials and surface charges have not been widely studied yet. Both polysulfone (PS) and polyether sulfone (PES) materials have low protein binding properties due to their hydrophilicity. However, it was demonstrated that PS could facilitate interactions between protein and lysed cells due to a higher negative charge density. Therefore, PS had a higher product retention than hollow fibers made of PES.
In addition to severe membrane fouling and the resulting low product sieving, the presence of protein aggregates in cell culture processes can also drastically reduce the product yield. In solution, monoclonal antibodies frequently aggregate to high molecular weight species (HMWS), a subset of aggregates consisting of dimers, trimers, or larger oligomers. Protein aggregation depends on multiple factors, including protein composition, protein expression, process conditions, and stability. The formation of HMWS can raise potential safety concerns, such as immunogenicity, and may also have significant impacts on the manufacturing process. A high proportion of HMW species is usually undesirable, as it increases the effort required for its elimination in the purification (e.g., number of purification steps) and, thus, leads to lower downstream process yields. Therefore, it is essential to monitor and understand protein aggregation in cell culture processes, especially in integrated continuous processes.
In this study, the magnetic levitation pump, which ensured the circulation of cell suspension with lower shear compared to peristaltic pumps, was evaluated in TFF perfusion cultures with the aim of assessing its performance in terms of product retention and its effects on cell culture performance (e.g., cell viability, growth rate, cell-specific productivities). The classical TFF mode was then modified to obtain a dynamic recirculation flow profile with pulsation of the TMP across the hollow fiber filter, with the hypothesis that this could reduce the reversible membrane fouling, and this was quantified. Another attempt to overcome the product sieving decay was then studied by applying a reverse flow (rTFF) with a second magnetic levitation pump installed on the retentate line of the TFF. The sieving performances of these systems were compared with those of a conventional alternating tangential flow filtration system. The sieving of mAb monomers and HMWS in these different modes of operations was also investigated.
METHODS
Cells
In this study, a recombinantly produced monoclonal antibody (mAb) was expressed in a CHO-K1 cell line with a glutamine synthetase (GS) expression system. Cells were thawed and routinely cultured in HyClone ActiPro cell culture medium (Cytiva) in shake flasks at 37°C, 5% CO2, and 120 rpm in a Minitron incubation shaker (Infors) before inoculation in the bioreactor.
Perfusion bioreactor design
Perfusion process runs were performed in glass stirred tank DASbox bioreactors (Eppendorf) with 200 mL working volume, according to the method previously described. The hollow fiber filter (HF) was a Xampler™ CFP-2-E-3MA 0.2 μm cartridge with 110 cm2 surface area and made of PES (Cytiva). The perfusion runs were performed either in alternating tangential flow (ATF), tangential flow filtration (TFF), or reverse tangential flow (rTFF) mode (Fig. 1(a). In ATF mode, the HF was connected to an XCell™ ATF2 (Repligen) cell retention device. The perfusion process in TFF mode was conducted with a low shear single-use pump (PuraLev® i30, Levitronix, Switzerland), based on the principle of magnetic levitation. The pump speed was controlled via the feedback signal from an ultrasonic clamp-on flow sensor (LFSC-i10X, Levitronix, Switzerland). In the TFF set up, two different recirculation flow profiles were tested. In a state-of-the-art approach, a constant TFF process (#TFF1) is run with a constant recirculation flow rate, delivering a constant shear rate across the hollow fiber (Fig. 1(b)). In processes where no permeate flux control (via pump) is applied and minimal or no fouling is present, the TMP stays constant over time. In a different TFF setup (#TFF2), a dynamic recirculation flow rate was applied by a recipe control in 2 cycles with defined run times, resulting in a step function of the flow rate and shear rate, respectively (Fig. 1(b)). The TMP in a dynamic TFF setup is therefore not constant anymore, but results in a pulsating behavior (wave function).
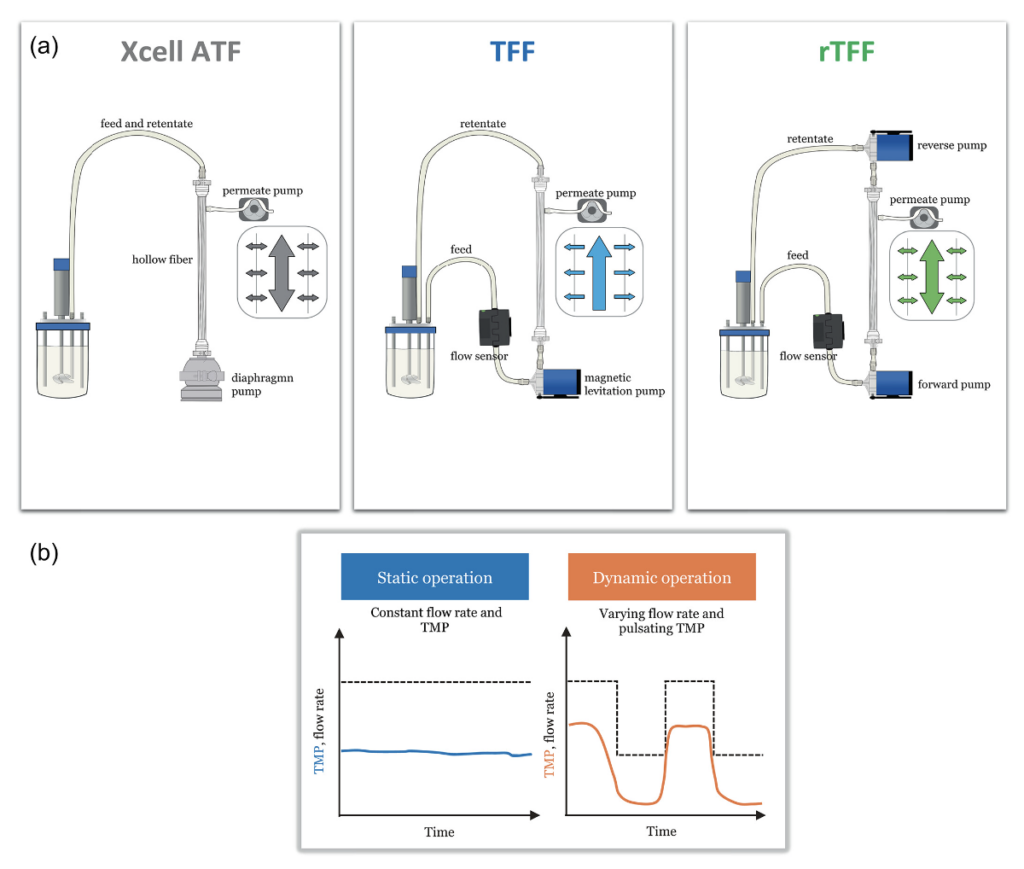
Figure 1
For the rTFF mode, a novel concept based on the application of reverse flow across the hollow fiber using two magnetically levitating pumps (see Results and Discussion) was demonstrated. An overview of operation conditions in the different ATF, TFF, and rTFF experiments is given in Table. The recirculation flow rate is given by the feedback signal from the attached flow sensor in the feed or retentate line; crossflow shear rates were calculated using Eq. (4) (Equations).
Table 1. Overview of the perfusion cultures operating conditions of recirculation flow rates, crossflow shear rates and modes of operation
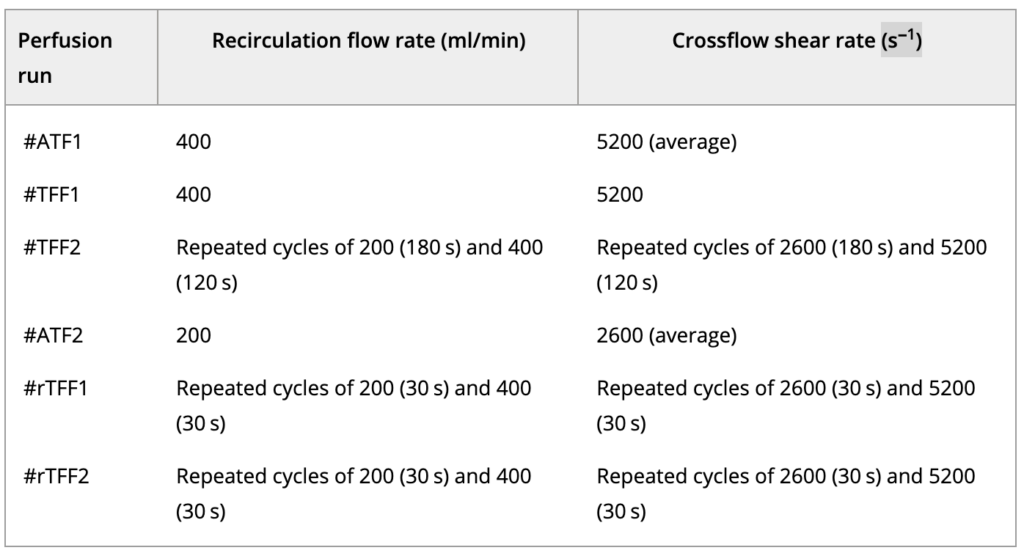
Perfusion cultures
The cultures were perfused using a medium composed of HyClone ActiPro media with HyClone Cell Boost 7a/7b (6%/1%) supplement (Cytiva) and 60 mM D-glucose; these were chosen based on a target cell specific glucose consumption of 1 pmol/cell/day to be achieved at steady-state with a set-point cell density of 60 × 106 cells/mL and a perfusion rate of 1 reactor volume per day (VVD), as previously described. The bioreactors were inoculated at a cell density of 3–5 × 106 cells/ml. The perfusion was initiated on the same day at 0.5 VVD by continuous supply of a feed medium into the bioreactor and removal of harvest from the permeate side of the hollow fiber (harvest sample). A perfusion rate of 0.5 VVD was maintained at this level until a density of 20 × 106 cells/ml and then it was linearly increased with the cell density to 1 VVD until the desired steady-state concentration set-point of 60 ± 10 × 106 cells/ml was reached. The working volume was kept constant at 200 mL by equal inflow and outflow rates with calibrated peristaltic pumps. Careful monitoring of feed, harvest (permeate), and bleed weights were used to adjust the pump flow rates, if necessary. Cell bleeding was applied by continuous removal of cell suspension from the bioreactor using a peristaltic pump to keep the biomass at the given setpoint. The selected bleed rate was equal to the average growth rate calculated within a sampling time interval (1 day) to maintain a constant cell density. At steady-state, the perfusion rate set-point was 1 VVD and the resulting cell specific perfusion rate (CSPR) was 17.8 ± 2.7 pL/cell/day. The perfusion rate was the sum of the rate of harvested supernatant (harvest rate) and the cell bleed (bleed rate); this value was also equal to the rate of added fresh medium, thus maintaining a constant volume in the bioreactor.
The setpoints of the temperature, dissolved oxygen concentration, and pH were 37 °C, 40%, and 7.0 ± 0.1, respectively. Six cultures (#ATF1, #TFF1, #TFF2, #ATF2, #rTFF1, #rTFF2) were performed in different perfusion operating conditions in terms of modes of operation (detailed information included in see Results and Discussion), crossflow shear rates of 2600 or 5200 s−1 in the fiber lumen, and recirculation flow rates in the ATF or TFF loop of 200 or 400 mL/min (Table 1).
Process analytics
Daily samples were taken from the culture to monitor the process parameters. To obtain the viable and total cell concentrations (VCC and TCC), viability and cell diameter were measured with a BioProfile FLEX analyzer (Nova Biomedical) and NORMA XS from iPRASENSE, respectively. Culture metabolites (glucose, glutamine, lactate, ammonium, and lactate dehydrogenase (LDH) levels) were obtained by Cedex Bio Analyzer (Roche Diagnostics). Antibody titers were determined with the IgG assay from Cedex Bio Analyzer (Roche Diagnostics).
Size exclusion chromatography
The detection of monomers aggregated and high molecular weight species (HMWS) was performed using size exclusion chromatography (SEC). A TSK Gel G3000SWXL, 7.8 × 300 mm2 size-exclusion column (Tosho Bioscience) mounted to a Waters 2695 HPLC unit was used, connected to a Waters 2996 UV detector for measurement of protein absorbance at 280 nm. A mobile phase of 100 mM sodium sulfate and 25 mM Na2HPO4 at pH 7.0 was used. Cell culture samples were centrifuged and filtrated using 0.22 μm filters. 20 μL samples were injected to the column and eluted for 60 min at a constant flow rate of 0.3 mL/min.
Equations


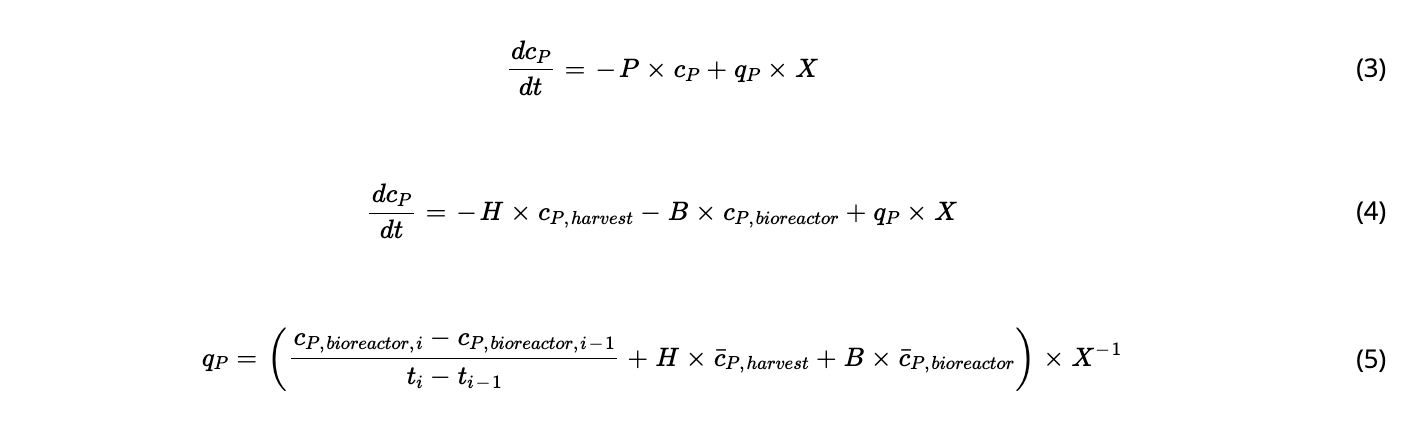





RESULTS AND DISCUSSION
Comparison between ATF and TFF systems
The process and cell culture performances between the two conventional systems, ATF and TFF operated unidirectionally with a magnetic levitation pump, were assessed in parallel small-scale perfusion bioreactor runs. A schematic representation of the flow circulation paths in the ATF and the TFF is given in Fig. 1(a), (b), respectively. Runs #ATF1 and #TFF1 were inoculated at a target cell concentration of 2.5 × 106 cells/ml and grown in batch mode for one day prior to the start of perfusion at 0.5 VVD. An uncommonly high crossflow shear rate of 5200 s−1 at a constant recirculation flow of 400 mL/min was applied to challenge the systems and test the feasibility of magnetic levitation pumps compared to ATF mode operated at 400 mL/min. The cell viability (second y-axis, right side) remained unaffected independently of the cell separation system throughout the experiments and stayed above 95% regardless of the high crossflow shear rate (Fig. 2(a)). The same was true for the cell specific growth rates shown in Fig. 2(b), which were in average similar using the TFF (0.25 d−1) or ATF systems (0.24 d−1)and didn’t change much over time. This indicated no detrimental impact of the high crossflow shear rate on the cell viability in the TFF system operated with a magnetic levitation pump, concordantly to Wang et al.’s report. During the cell density increase phase, the perfusion rate was gradually increased according to the cell growth and nutrient consumption (target cell specific glucose consumption of 1 pmol/cell/day) and maintained at a constant perfusion rate of 1 VVD throughout the experiments. The cell density (first y-axis, left side) was maintained at 60 ± 10 × 106 cells/ml in both experiments (Fig. 2(a)), with a constant cell bleed corresponding to the average growth rate within a sampling time interval (1 day). The perfusion rate, harvest rate, and cell bleed rate were stabilized from day 7 at steady-state until the end of the cultures (Fig. 2(c)). The CSPR was decreased from 80 (ramp up phase) to 15–20 pL/cell/day (steady-state) to reduce the medium consumption and increase the product titer. A constant CSPR could be maintained throughout the steady-state phase (Fig. 2(d)). Metabolite profiles of L-glutamine, ammonium, lactate, and glucose concentrations of runs #ATF1, #TFF1, and #TFF2 perfusion cultures quantified from the perfusion harvest samples are depicted in Supporting Information, Fig. S1.
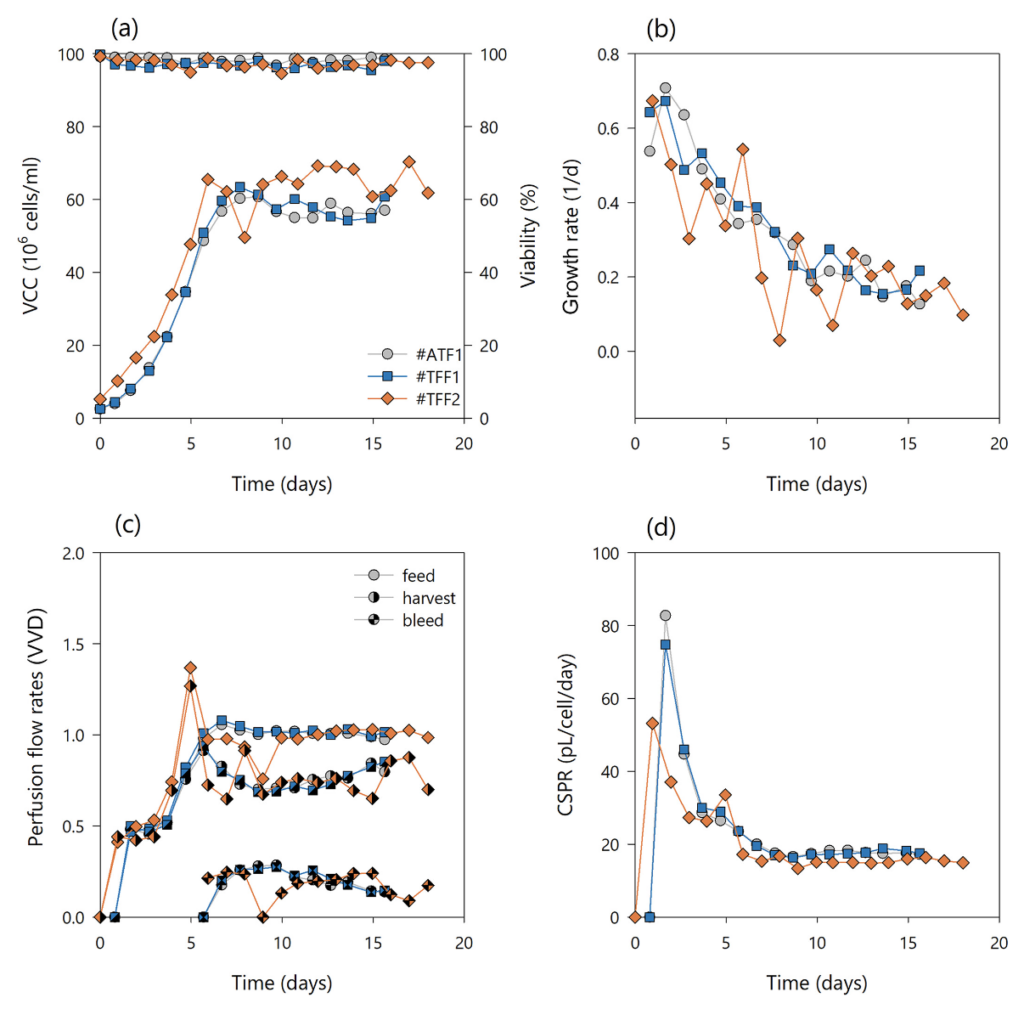
Figure 2
Although similar VCC and viability patterns were achieved, different mAb titers in the bioreactor and in the harvest stream were observed between runs #ATF1 and #TFF1 (Fig. 3(a)). In #ATF1, the majority of the product passed the hollow fiber, reaching an average mAb titer in the bioreactor of 1000 mg/L and of 900 mg/L in the harvest. In #TFF1, the mAb titer increased to 1400 mg/L in the bioreactor, while only 700 mg/L was present on average in the harvest stream (Fig. 3(b)). However, the cell specific productivities were comparable in both experiments (Fig. 3(c)). The overall mass balance within 16 days production for #ATF1 was 73.6% mAb in the harvest, 17.8% mAb loss in the cell bleed, and 8.5% residual mAb in the bioreactor on day 16, whereas run #TFF1 resulted in 60.9% mAb in the harvest, 27% mAb loss in the cell bleed, and 12.1% residual mAb in the bioreactor. Thus, the responsible parameter for the difference in mAb titer between bioreactor and harvest in this case was the sieving. In #ATF1, the product was harvested with a sieving coefficient of 88–95%, while in #TFF1, the sieving decreased to 52% over time (Fig. 3(d)). Although membrane fouling can be generally reduced at high crossflow shear rates by increasing the tangential flow, the problem of product retention and restriction of TFF operation could not be circumvented in the #TFF1 experiment. The results of constant final sieving and lower sieving profiles with increased shear rates are in line with previously published TFF perfusion data. However, in this study, membrane fouling and subsequent product sieving could not be linked to cell viability and cell debris, as previous studies reported. Usually, TMP is a constant variable during filtration processes that is highly dependent on the dynamic flow and geometry of tubing. If no permeate control is applied and membrane fouling increases during a process, this leads to a decrease in flux caused by increased resistance to the mass transport through the membrane. However, in this study, membrane fouling may increase resistance to fluid flow and manifest in a potential increase in TMP as a constant permeate flux is applied by a pump to keep the bioreactor volume constant by controlling ingoing and outgoing flow rates. Nonetheless, the full fouling phenomenon and impact on TMP is not clear, since the experiments were conducted without pressure monitoring on the feed, retentate, and permeate side. Although the sieving profile was rather different between #ATF1 and #TFF1, no detrimental effects on cell growth, viability, cell specific productivities, and other process parameters were observed using magnetic levitation pumps.
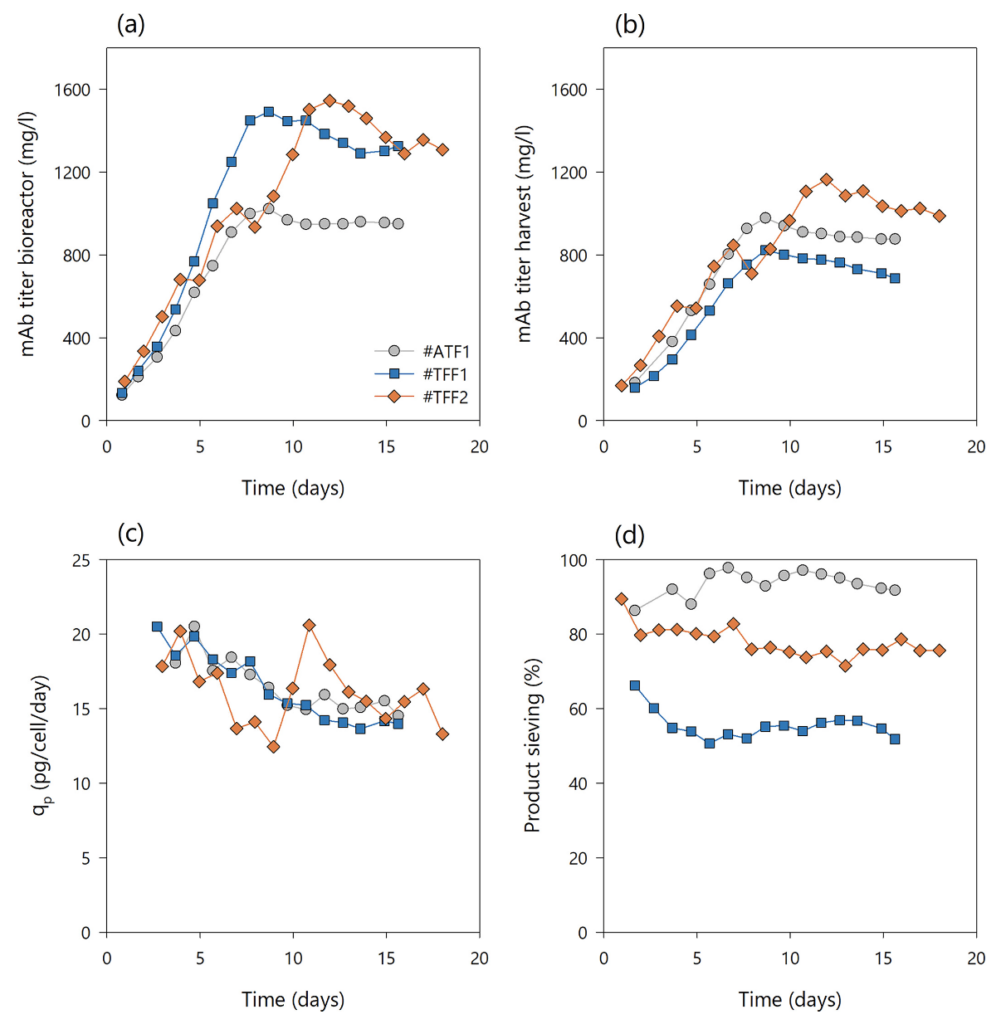
Figure 3
Improvement of product sieving decay through dynamic TFF mode
The #TFF1 perfusion process achieved similar process characteristics compared to #ATF1 when applying a head-to-head comparison, except that, at high shear rates, the #TFF1 run resulted in poor product sieving profiles and a lower yield. An additional experiment was carried out to improve the rapid product sieving decay in TFF operation mode and to increase the product yield in harvest without the need to change the hollow fiber geometry, as previously suggested. The process parameters and conditions were the same as those used for the previous runs (#ATF1 and #TFF1), except for the recirculation flow rate and associated crossflow shear rate. In run #TFF2, a dynamic recirculation flow rate of 200 and 400 mL/min was applied by recipe control in 2 cycles. Therefore, in phase 1, a shear rate of 2600 s−1 was applied for 180 s and in phase 2 a shear rate of 5200 s−1 for 120 s. This caused the TMP to run cyclically throughout the course of the experiment, generating dynamic flow behavior. As expected, no difference in growth rate and viability of the culture (>94%) was observed between the different setups; hence, dynamic flow was not an issue for the cells (Fig. 2(a), (b)). The flow set points of perfusion, bleed, and harvest rates were performed similarly (Fig. 2(c)) and, therefore, had the same CSPR progression as #ATF1 and #TFF1 (Fig. 2(d)). Metabolite profiles of L-glutamine, ammonium, lactate, and glucose concentrations of runs #ATF2, #rTFF1, and #rTFF2 perfusion cultures quantified from the perfusion harvest samples are depicted in Supporting Information, Fig. S2. The mAb titer in bioreactor and harvest increased until steady-state, reaching up to 1502 and 1164 mg/L, respectively, with similar cell specific productivities between 14 and 20 pg/cell/day (Fig. 3(a)–(c)). It was observed that the product sieving was drastically increased by around 25–30% compared to #TFF1 using this dynamic recirculation flow. Figure 3(d) shows that product sieving had already dropped to 80% by the third day, but remained rather stable throughout the experiment, with lowest sieving coefficients of 71–75%. No higher particle count or background level was observed in the #TFF1 run compared to the #TFF2 run, which would indicate a higher cell lysis rate and contribute to poorer product sieving (data not shown). Moreover, the growth rates and viabilities of both runs were rather comparable. Thus, the improvement in system performance can be attributed to one cause, namely the dynamic recirculation. It is assumed that the continuous change in recirculation flow through the hollow fiber creates a fluctuating, pulsating flow that counteracts the formation of a cake layer. Moreover, the change in local TMP could be responsible for a different product retention behavior. This rationale was confirmed by off-line membrane flux testing and TMP measurements using RO-water and cell suspension (data not shown). A recent study investigated and confirmed a similar performance using modeling approaches by yeast cultures and BSA as permeating solute. Thus, it was shown that the use of dynamic recirculation flow was preferable to constant flow in tangential flow filtration systems. Product sieving performance could be significantly improved, while the cell performance remained unaffected.
Reverse TFF (rTFF) flow alleviates product sieving
It has been claimed that the commercially available alternating tangential flow (ATF) systems (Repligen, USA) achieve better product recovery11 and, therefore, they have gained popularity in recent years due to their superior performance in high cell density cultures and integrated continuous bioproduction. It is assumed that backwashing of the filter by a periodic pressure and exhaust cycle via a diaphragm pump leads to a change in hydrodynamics and, thus, to a partial regeneration of the hollow fiber during processing. Although ATF has become a versatile alternative to unidirectional TFF technology, it may have some drawbacks arising from limited setting options and the complexity of ATF controllers. In addition, the large retention volume of the diaphragm pump is not advantageous for performing small-scale perfusion processes, as it results in major hold-up volume differences in the bioreactor.
A novel concept for better product recovery in TFF systems in comparison with commercial ATF technology by using two alternating magnetic levitating pumps was demonstrated. The pumps generated a low shear reflux (rTFF) across the hollow fiber (on the same principle as ATF), allowing for long process duration without increased membrane fouling. This concept was tested in two experiments (#rTFF1 and #rTFF2) and accordingly compared with data obtained using commercial ATF systems (Table 1). The cycle time of both pumps was aligned to the ATF pressure and exhaust cycle time of 60 s and was approximately 30 s at 200 mL/min flow rate for each pump, resulting in a total of 60 s cycle time for both pumps. Since the dead volume in the recirculation line was rather oversized compared to the bioreactor volume, dynamic flow was also used in the rTFF systems. This ensured that the system was periodically flushed at a high flow rate to minimize air bubbles in the system and, thus, prevent negative effects on the cells. The process performance and cell behavior between the two systems, ATF and alternating rTFF, were assessed in short-term parallel experiments, #ATF2 and #rTFF1 (Fig. 4). The applicability and long-term performance was then assessed in a replicate experiment of #rTFF1 (#rTFF2). The cultures were again grown to target VCC (first y-axis, left side), at which time a cell bleed was initiated to maintain steady state. The cultures had the same growth and viability profiles (second y-axis, right side) as the previous ATF and TFF cultures, #ATF1, #TFF1, and #TFF2 (Fig. 4(a), (b)). The perfusion, bleed, and harvest flow rates were controlled according to prior runs (Figs 2(c) and 4(c)) and resulted in similar CSPR profiles (Fig. 4(d)). Interestingly, switching to a reverse flow with two low shear levitating pumps eliminated the differences in product sieving between commercial ATF and TFF (Fig. 5). Figure 5(a), (b) shows that the mAb titers in the bioreactor and harvest had similar values for each respective run, indicating a high product sieving coefficient in the rTFF runs comparable to that of the ATF runs. The total amount of mAb produced in #rTFF2 run was clearly lower than that in runs #ATF2 and #rTFF1. This may be attributed to the fact that a higher cell generation number was used for the #rTFF2 experiment, resulting in a decrease in cell-specific productivity (Fig. 5(c)); this is possibly due to epigenetic drifts and cell ageing,41 as similar behavior has been observed with this cell line in our lab. The experiments #ATF1, #TFF1, #TFF2, #rTFF1, and #ATF2 were all conducted with the same cell generation number (cell age) and their similar behaviors in terms of average growth rates and cell-specific productivities (in steady-state) can be seen in Supporting Information, Fig. S3. Only run #rTFF2 showed lower values due to the older age of the cells. Despite this decrease in the total amount of mAb produced in the #rTFF2 run, the curves of mAb titers in the bioreactor and harvest were similar. Product sieving remained >89% in both rTFF runs compared to 88% in #ATF1 and #ATF2, indicating that sieving was significantly alleviated and comparable to that of commercial ATF (Fig. 5(d)). Therefore, the reflux application of two TFF systems operated with two magnetic levitation pumps showed the same performance as a commercial ATF system. The biggest advantage of the rTFF set up is the constant bioreactor hold-up volume that can be achieved compared to ATF processes, where the working volume in the bioreactor is dynamic due to pressure and exhaust gas cycles. Moreover, rTFF control offers more alternatives in terms of cycle times, dynamic recirculation flow rates, and shear rates than using the ATF controller. However, this study focused on the proof of principle of rTFF technology in cell culture and does not include extensive testing of cycle times, shear rates, or their effects on the process and screening performance in such a system.
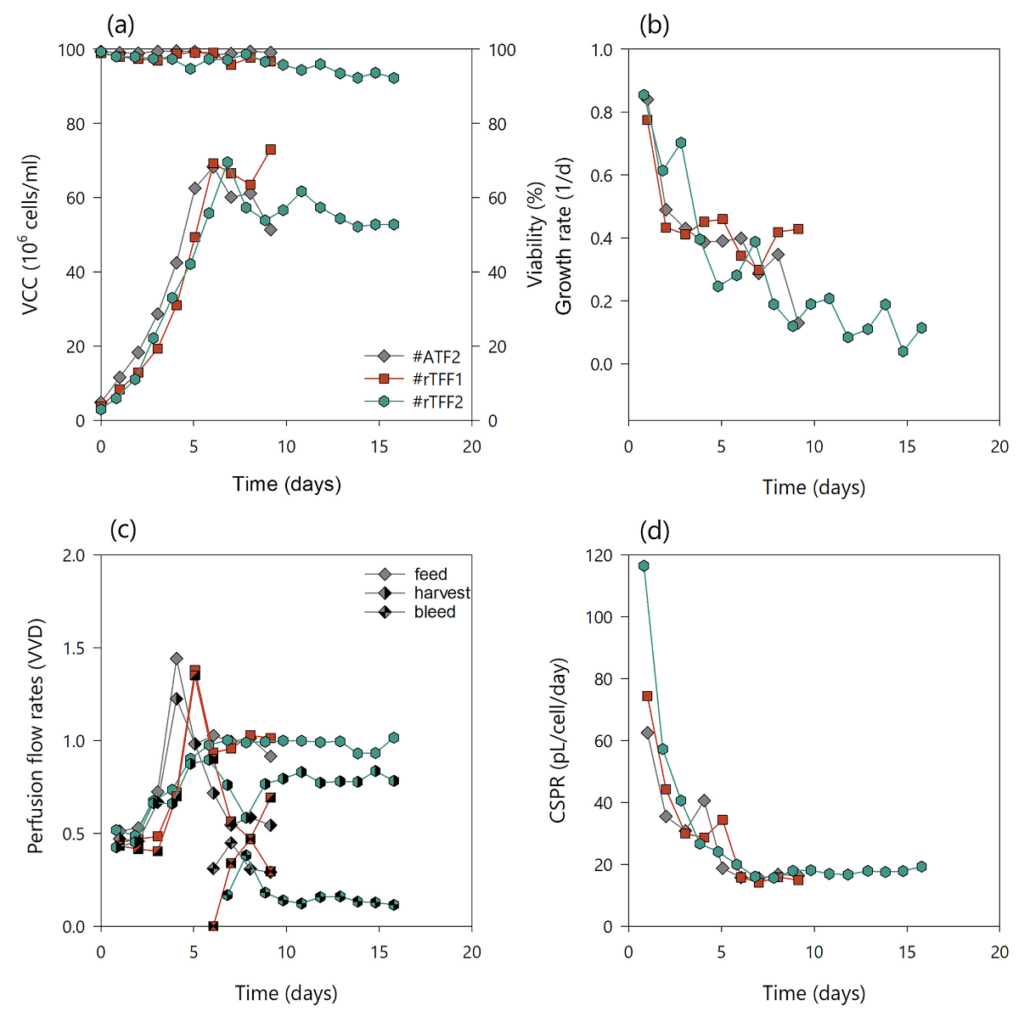
Figure 4
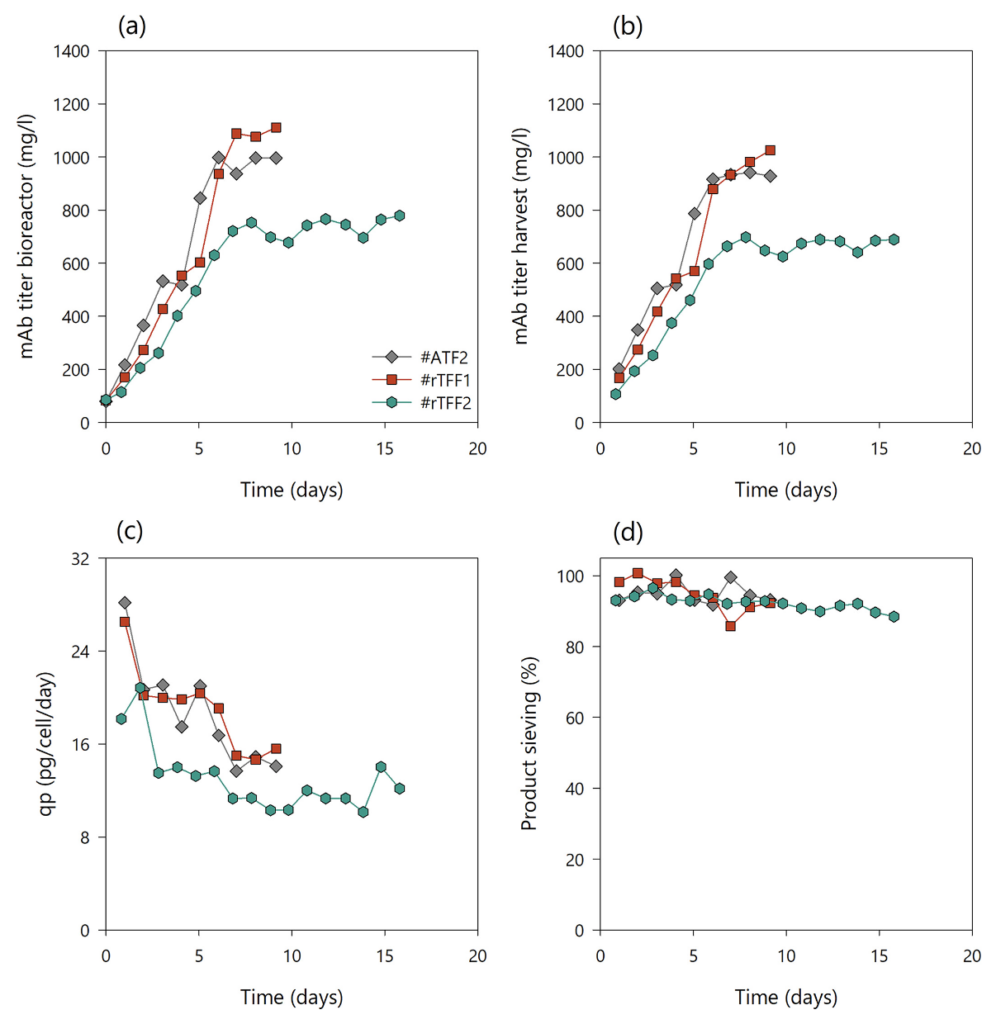
Investigation of protein impurities
Within this study, several techniques were demonstrated to address the issue of product retention in TFF perfusion cultures and to increase product yield. Another aspect that reduces product purity is the presence of LMWS, HMWS, and aggregates in the cell culture process. Increased HMWS concentration decreases product yield and has the potential of increasing the complexity of downstream processing. This may impact resin choice and influence the required number of purification steps. The potential to affect product stability and safety is critical to the manufacturing process. Therefore, it would be beneficial to understand the transport and presence of HMWS in the downstream capture unit, especially in integrated continuous biomanufacturing (ICB).
To monitor protein aggregation, SEC chromatography measurements were performed directly from supernatant samples without pretreatment or purification. The used method was capable of resolving HMWS from monomer and cell broth constituents, allowing for the analysis of HMWS contents in the perfusion bioreactor and harvest samples. To reduce the resin fouling, a cleaning sample and blank was run after ten injected cell culture samples. Figure 6 shows the chromatograms associated with the analysis of cell culture samples from the bioreactor (green) and harvest (blue) using SEC. Harvest chromatograms are shown with 0.15 and 0.2 AU off-set for clarity reasons. The monomer peak has a retention time of approximately 28 min and the HMWS peaks retain from 17 to 24 min. Peaks remaining later than 33 min are associated with LMWS, media, and buffer constituents. The SEC chromatograms at the end of the culture (day 15) are shown for runs #ATF1 (Fig. 6(a)), #TFF1 (Fig. 6(c)), and #rTFF2 (Fig. 6(e)). The peak heights of the monomers in the bioreactor and harvest samples are similar, but the eluted HMWS peaks differ significantly in #ATF1 (Fig. 6(b)) and #TFF1 (Fig. 6(d)). While the majority of HMWS peaks are found in the bioreactor samples, only a portion is transported through the hollow fiber into the harvest in the ATF and rTFF (Fig. 6(f)). Peak heights in #rTFF2 (Fig. 6(e), (f)) are significantly smaller than those in #ATF1 and #TFF2 due to lower mAb concentration in both bioreactor and harvest samples (see Reverse TFF (rTFF) flow alleviates product sieving). In #TFF1 (Fig. 6(d)), a large fraction of the HMWS is retained so that the HMWS peaks in the bioreactor at the end of the culture – day 15 (gray line) – are significantly larger than those at the beginning of the steady state – day 7 (black line). Mainly, 3 different HMWS peaks with retention times of 17, 18.5, and 24 min could be identified. However, the main difference is the retention of HMW species in the TFF harvest. A certain protein amount is retained in the bioreactor due to low sieving, and this affects the transport of HMW species into the harvest; almost no HMWS peaks are found in the harvest sample, neither at the beginning (day 7) nor at the end of the culture (day 15). One explanation could be the flux reversal in ATF and rTFF that is responsible for backwashing proteins deposited as a gel layer on the membrane surface (when reversible fouling is present). This can result in proteins of certain sizes and molecular weights being more likely to pass than in TFF mode, where unidirectional flow is applied. This is particularly evident when the sieving coefficient is low and a cake layer forms over time, acting as an additional barrier.
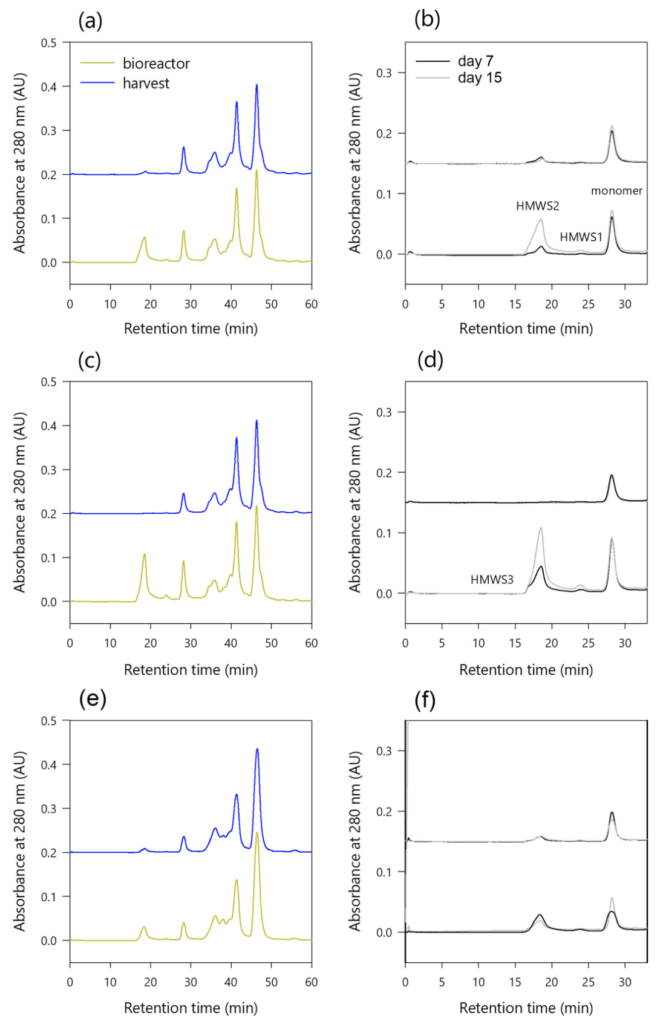
Figure 6
Product recovery in different perfusion systems
An investigation on antibody purity and the amount of high molecular weight impurities was performed throughout the steady state phases of 4 different perfusion processes (#ATF1, #TFF1, #TFF2, and #rTFF2). After monomer, HMWS, and LMWS peak integration, the apparent antibody purity was calculated from the relation between the monomer and total peak area. Figure 7(a) shows the trend of apparent antibody purity (or relative amount of monomer in percentage) in the bioreactor (solid line) and the harvest (dashed line) for the different perfusion process set ups. The amount of protein monomers in the bioreactor decreased over time in all the experiments and resulted in 38–50% monomer at the end of the culture. In contrast, the amount of monomer in the perfusion harvest remained constant. A lower purity was measured when alternating flow was applied (#ATF1, #rTFF2) than in the TFF processes (#TFF1 and #TFF2, respectively). Accordingly, both TFF processes resulted in a higher protein purity in the harvest than ATF and rTFF.
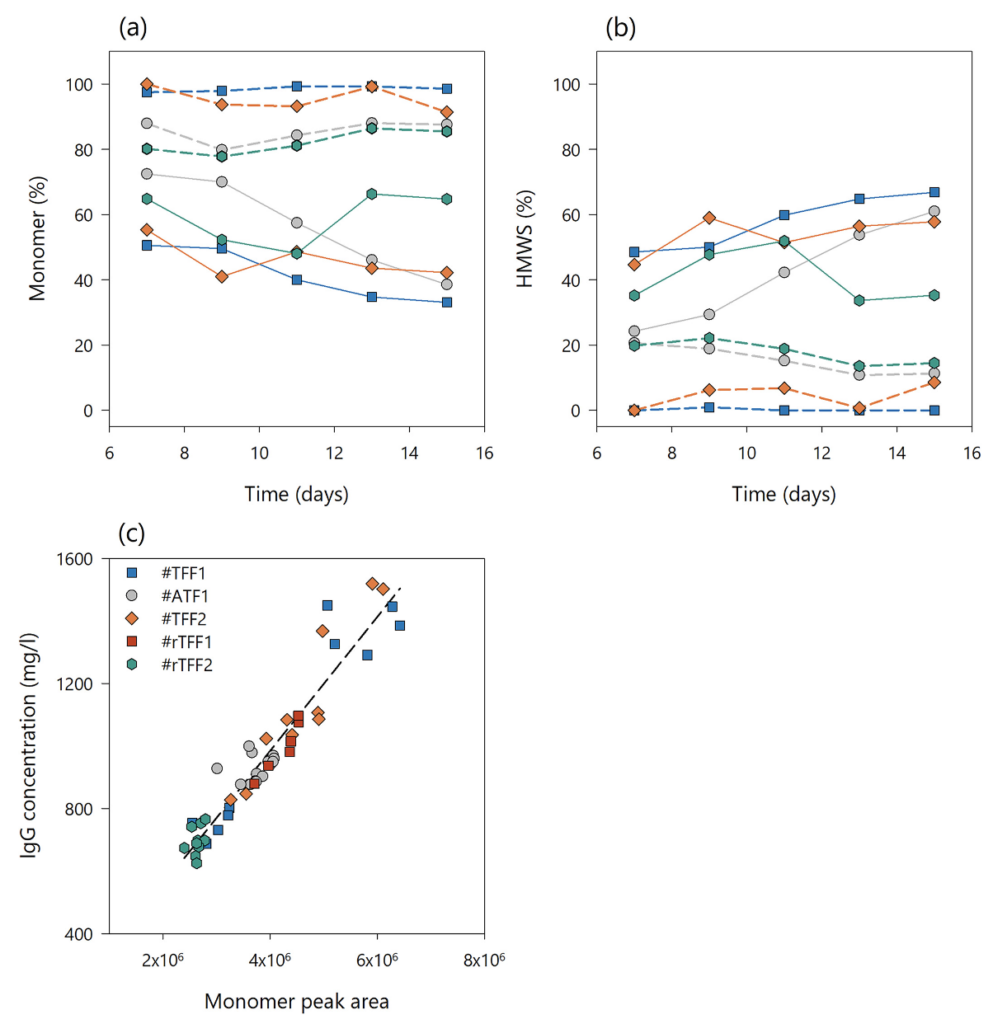
Figure 7
Similar to that, the amount of HMWS in relation to the total peak area was monitored in the aforementioned process set ups (Fig. 7(b)). The amount of HMW impurities in the bioreactor increased over time in all the runs, with a maximum of about 60% in run #TFF1. However, the total amount of HMWS in both TFF harvests resulted in less than 5% over 10 days steady state production. On the other hand, ATF and rTFF operation modes resulted in a similar HMWS profile of 15–20% throughout the process. Thus, it can be concluded that membrane fouling and product retention (related to the hollow fiber set up) have a direct impact not only on the overall product yield, but also on the product purity in the perfusion harvest. The data show that higher product retention with the TFF set up results in a lower product yield but in higher protein purity than with the alternating tangential flow set up. Although flow reversal in ATF and rTFF modes increases product transmission through the hollow fiber filter, it also facilitates the passage of HMWS through this filter. This may increase the complexity of the downstream unit operation, which must deal with higher impurity levels from ATF or rTFF perfusion harvest than from TFF. These benefits become overshadowed, however, if the amount of protein one can produce and recover is drastically reduced as a consequence of using TFF over ATF.
The relevant monomer, aggregate, and HMWS related peaks were evaluated in all the chromatograms. For monomer, the 280 nm monomer peak response was found to behave in a linear fashion and intercept near the origin against the measured IgG concentration. The resultant linear regression analysis for data points from 5 different perfusion processes is shown in Fig. 7(c). A correlation with the amount of IgG monomer at 280 nm was demonstrated for several IgG concentrations tested in the range of 600–1500 mg/L. From this, it is possible to relate the product sieving coefficient (calculated from IgG concentrations) to the total percentage of HMWS.
Passage of HMWS to harvest is sieving dependent
To evaluate the impact of different perfusion modes on integrated continuous bioprocessing, the average monomer and HMWS content in the steady state phases of each bioreactor run was calculated (n = 5). As previously demonstrated, the alternating modes, ATF and rTFF, resulted in higher apparent antibody purity in the bioreactor but lower monomer content in the harvest stream with 86 ± 3.6% in #ATF1 and 82 ± 4% in #rTFF2 (Fig. 8(a)). In comparison to that, a higher harvest purity was achieved in both TFF processes with 99% ± 0.8% in #TFF1 and 96 ± 6% in #TFF2. Compared to that, Fig. 8(b) shows the average HMWS content at steady state with higher content in TFF-based bioreactors, but minimal to no HMWS presence in the harvest stream (0.2 ± 0.4% in #TFF1 and 4 ± 4% in #TFF2). The total amount of HMWS in the bioreactor and harvest of #ATF1 and #rTFF2 were similar (#ATF1, 15 ± 4.4%; and #rTFF2, 18 ± 4%), which might be due to the fact that they also showed comparable product sieving performances (Fig. 8(c)).
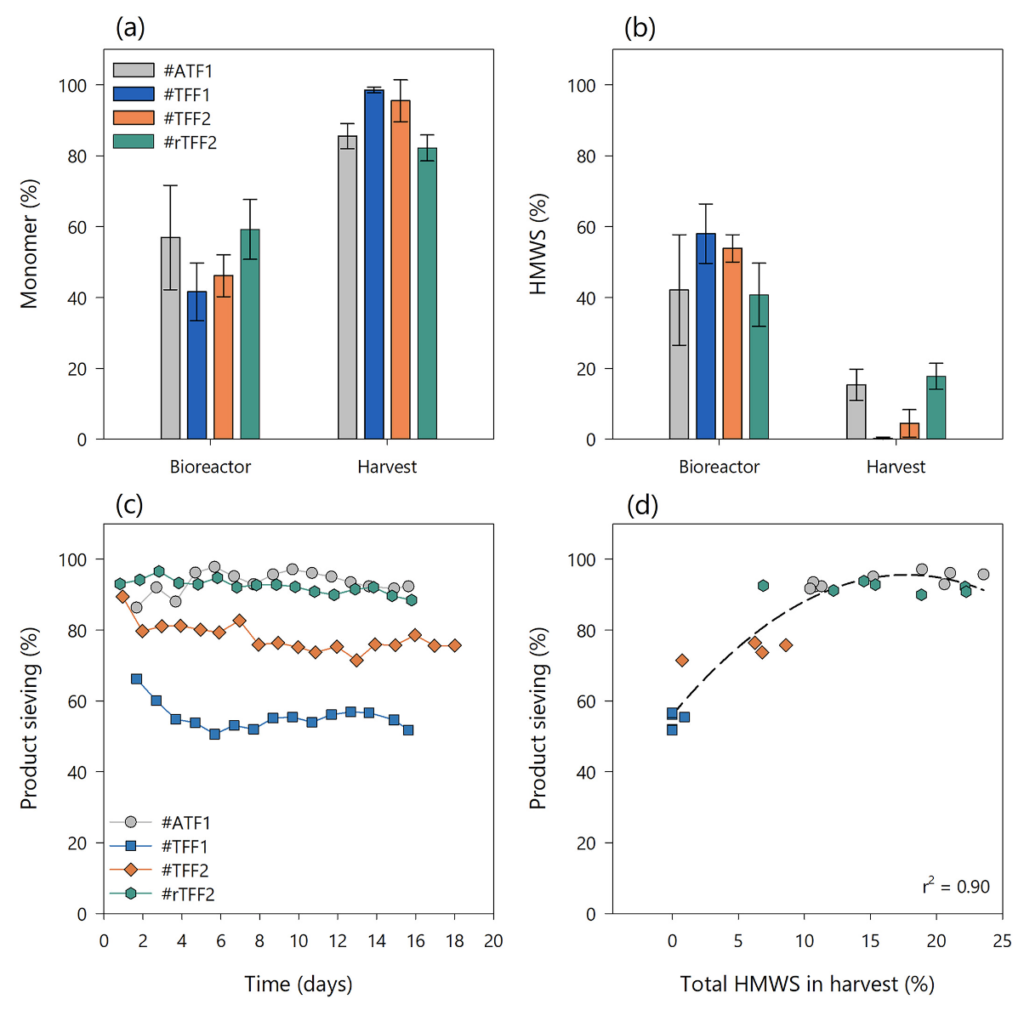
Figure 8
Figure 8(c) compares the product sieving profiles of the four different perfusion runs. From these data, we raised the hypothesis that the product sieving coefficient and the mode of operation could be the driving factors for the amount of high molecular weight species in the perfusion harvest and, thus, for product purity. For example, comparing the sieving profiles of both TFF experiments shows that lower product sieving coefficients (#TFF1) transfer less HMWS to the perfusion harvest and vice versa. One reason for this could be that the composition of the cake layer formed during membrane fouling processes is less permeable to larger molecules (e.g., dimers, trimers, and aggregates) than to monomeric proteins. Therefore, it can be assumed that there is a threshold of HMWS transmission when the sieving decreases to a certain range or when the membrane fouling increases to a high level. We believe that the product sieving coefficient can be correlated with the passage of HMWS into the perfusion harvest.
Figure 8(d) shows that the product sieving is related to the total amount of HMWS in the perfusion harvest at different time points in a non-linear manner. A quadratic regression was successfully used to model the relationship between the two sets of parameters (r2 = 0.9). This relationship enables scientists to estimate and monitor the apparent product purity of perfusion harvest via the at-line measured product concentration and subsequently calculated product sieving coefficients. This can be particularly helpful and beneficial in integrated continuous bioprocesses when no on-line or real-time monitoring of protein impurities or aggregates is accessible.
Membrane fouling and subsequent (product) retention are major challenges in perfusion bioprocesses. Several approaches have already been established to reduce fouling principles. In particular, it has been demonstrated that filters with larger pore sizes9 or wide-surface pore membranes are capable of overcoming the product sieving decay in perfusion cell culture. Using this approach, product sieving profiles of TFF perfusion systems could be obtained that were similar to those of ATF systems and that offered an opportunity for long term integrated continuous bioprocessing. The beneficial effects of alternating flow to support high cell densities and mitigate fouling has been extensively studied. The main advantage is the ability to flush away particles from the membrane surface of the hollow fibers to minimize product retention when reversible fouling is present.15, 20, 24, 26, 43 According to the state of the art, this is done via a diaphragm pump together with a controller. The application of a reverse flow across the membrane, along with studies on the relationship between filtration fluxes and retention, have both revealed that flux as a key parameter to minimize or prevent fouling.16, 20 It has been shown that the use of rTFF principles with a magnetically levitating pump does not affect cell growth, viability, or cell specific productivity; therefore, this process can be considered a versatile alternative to commercial ATF systems.
CONCLUSION
The applicability of low shear TFF operation in small scale perfusion bioreactors for continuous antibody production was shown using magnetic levitation pumps. We provided two solutions for the product sieving phenomenon in TFF-based perfusion cultures. By applying a dynamic recirculation flow across the hollow fibers, a pulsating TMP facilitated product passage by 25% compared to a constant unidirectional flow of TFF. More notably, a total improvement of the product retention phenomenon in TFF perfusion cell culture was shown by a novel concept based on the application of reverse flow across the hollow fiber using two magnetically levitating pumps (rTFF). Installing a second pump on the retentate side of the fiber and thereby using forward and reverse flows alleviated product passage close to 100% for the entire process. Performance was tested at constant and cycling shear rates for different process set ups. As a result, the cells remained unaffected by shear stress and showed similar growth and production behaviors in all systems, regardless of shear rate and mode of operation (ATF, (r)TFF). This comprehensive solution for the application of reverse flow in TFF yielded a robust, easy to establish, and similar performing perfusion process compared to conventional ATF systems with the advantage of operating at a constant bioreactor hold-up volume.
Moreover, the data demonstrated that membrane fouling in hollow fiber-based perfusion processes can affect not only the product yield but also the product purity. Overall, the results showed that unidirectional TFF mode had a greater retention rate than dynamic and reverse TFF – resulting in a poorer product yield. Accordingly, a TFF process leads to a purer harvest stream due to lower HMW content (higher antibody purity) but to a lower product yield because antibodies are retained in the bioreactor. It was shown that the amount of HMW impurities in perfusion harvest differ between uni- and bidirectional flows. The total product yield is more affected by higher levels of protein impurities in the harvest stream in ATF and rTFF modes than in TFF mode. Therefore, the higher product yield achieved in alternating tangential flow filtration systems does not concomitantly result in higher product purity. As a result, the purity of the material being processed would differ depending on the perfusion process set up and is, therefore, a challenge for the downstream unit. A correlation could be made between the product sieving coefficient and the HMWS levels to infer the degree of impurities originating from different set ups. This provides an important opportunity for the operation of an integrated continuous bioprocess where this correlation can be used to adjust the downstream purification step accordingly based on the sieving coefficient. As a result, consistent product purity or the separation of aggregates or HMW impurities can be achieved. Impacts on the purification process with regards to different HMWS levels and species when considering a fully integrated continuous process should still be considered in future studies.
AUTHOR CONTRIBUTIONS
MP, HS, BS, AJ, and VC contributed to conception and design of the study. MP and HS performed the experiments. MP analyzed the data. MP drafted the manuscript in consultation with HS, BS, AJ, and VC. All authors contributed to manuscript revision, read, and approved the submitted version.
ACKNOWLEDGEMENTS
Hubert Schwarz is supported by the Competence Centre AdBIOPRO funded by Vinnova (AdBIOPRO diarie 2016-05181). The work was supported by funding from the Austrian Research Promotion Agency (Frontrunner Initiative, 878717). The authors would like to thank Johanna Pechan from the CETEG group at Dept. of Industrial Biotechnology, KTH, for the practical contribution to this work. The authors also thank the Levitronix Team, GmbH, Zurich, Knut Kuss, Antony Sibilia, and Christopher Miggitsch for their helpful suggestions and discussions within this work.
CONFLICT OF INTEREST
Authors MP and BS are employed by Bilfinger Life Science GmbH. The remaining authors declare that the research was conducted in the absence of any commercial or financial relationships that could be construed as a potential conflict of interest. The experimental work was not financially supported by Levitronix GmbH.